Characterization of Rhesus Macaque lung-resident multipotent stromal cells
Cell Gene Therapy Insights 2016; 2(1), 49-72.
10.18609.cgti.2016.002
Ryan W Bonvillain, Michelle E Scarritt, Nicholas C Pashos, Deborah E Sullivan, Aline M Betancourt, Fern Tsien, Ayesha P Umrigar, Andrew M Hoffman and Bruce A Bunnell Research Article Current lung regeneration strategies focus on the airway epithelium, pulmonary endothelium, and their putative progenitors; however, the supportive pulmonary stroma has been widely overlooked. Tissue-resident multipotent […]
Ryan W Bonvillain, Michelle E Scarritt, Nicholas C Pashos, Deborah E Sullivan, Aline M Betancourt, Fern Tsien, Ayesha P Umrigar, Andrew M Hoffman and Bruce A Bunnell
Research Article
Current lung regeneration strategies focus on the airway epithelium, pulmonary endothelium, and their putative progenitors; however, the supportive pulmonary stroma has been widely overlooked. Tissue-resident multipotent mesenchymal stem cells have recently been identified in a host of mammalian tissues including the lungs. In this report, we isolated lung-resident mesenchymal stromal cells from the non-human primate, rhesus macaque. These cells expressed cell surface markers consistent with those expressed by mesenchymal stem cells isolated from bone marrow, and they also demonstrated variable degrees of differentiation into bone, adipose, and cartilage. When cultured on Matrigel, the cells formed tubules, and when cultured on decellularized macaque lung scaffolds, the cells attached to the matrix with minimal apoptosis observed. Given the ease of isolation, characteristics similar to other mesenchymal stem cells, and engraftment to decellularized lung scaffolds, lung-resident mesenchymal stromal cells may be valuable in tissue engineering applications as well as other therapeutic strategies that employ mesenchymal stem cells.
Submitted for Review: Jul 27 2015; Published: Mar 21 2016 ; DOI: 10.18609.cgti.2016.002
Citation: Cell Gene Therapy Insights 2016; 2(1), 49-72.
It is becoming increasingly evident that multipotent stromal cells, also known as mesenchymal stem cells (MSCs), reside in most, if not all, adult tissues [1–4]. However, their in vivo function remains poorly understood. In general, MSCs are characterized in vitro by the simple criteria of adherence to virgin tissue culture plastic, spindle shape, formation of colonies (self-renewal), expression of cell surface markers associated with MSCs (CD90, CD105, CD29, CD73) and lack of hematopoietic markers (CD45, CD34, CD11b, CD3), and differentiation into multiple lineages [5–7]. While the field of MSC research has focused largely on bone marrow- and adipose-derived MSCs (BMSCs and ASCs, respectively), cells with similar characteristics have been isolated from various tissues including the lungs of multiple human and animal subjects via bronchioalveolar lavage (BAL), fluorescence-activated cell sorting (FACS) of cells that efflux vital dyes, and explant outgrowth culture [1,8–11]. Similarly to their bone marrow and adipose counterparts, the resulting lung MSCs (L-MSCs) demonstrate robust and extended proliferation in vitro while maintaining their stem-like characteristics including multipotency. Moreover, studies of L-MSCs have shown that these cells possess anti-inflammatory and immune-suppressive properties [12,13] and are protective in animal models of lung injury similar to the BMSCs and ASCs [8,14].
During organogenesis, the lung mesenchyme plays a critical role in directing the complex branching morphology of the airway as well as the differentiation of pulmonary epithelial cells [15–17]. Mounting evidence suggests that the adult lung mesenchyme retains the function of organizing epithelia perhaps via recruitment of progenitor populations during normal epithelial maintenance or by differentiating into myofibroblasts during injury, repair, and/or remodeling in response to microenvironmental cues [18,19]. Given the necessity of the lung mesenchyme and what has been learned about MSC populations, it is likely that L-MSCs contribute to the functional mesenchymal population in the lung and may play an important role in lung health throughout the lifetime of an individual.
Given the indispensable role of the mesenchyme in lung development and maintenance, it is logical to conclude that lung bioengineering applications will have to employ these mesenchymal stromal cells for efficient epithelialization of the airway and endothelialization of the vasculature in the bioengineered organ. A non-human primate (NHP) model for lung bioengineering utilizing natural organ scaffolds produced by decellularization of rhesus macaque (Macaca mulatta) lungs has been established by our group [20,21]. In light of the evidence for the supportive role of L-MSCs in lung development, healing, and regeneration, we sought to identify and characterize a population of L-MSCs in the rhesus macaque and to investigate their potential for contributing to lung scaffold recellularization. The data presented in this report complement our previous findings with NHP-derived BMSCs and ASCs toward the combined goal of optimizing the production of bioartificial lungs in this important pre-clinical model.
Methodology
Lung tissue samples
Lung tissues were obtained from rhesus macaques housed at the Tulane National Primate Research Center according to institutional protocols. Animals from which tissues were taken were either used by other investigators as normal experimental controls (obtained with permission) or were sacrificed due to unresolvable medical issues including chronic diarrhea, self-mutilation, and failure to thrive. A skilled prosector removed the lung tissue during necropsy.
Explant culture & collection of outgrowth cells
Lung lobes from necropsy were collected in ice-cold PBS (no Ca2+/+) containing 5 mM EDTA to prevent blood coagulation. The tissue was perfused through available pulmonary arterioles or venules with PBS containing 50 U/ml heparin and 5 µg/ml sodium nitroprusside (SNP) to remove excess blood from the pulmonary capillary bed. Generation of lung explant cultures was performed according to modifications to a previous published protocol [8]. Briefly, the perfused tissue was sliced into strips of ~0.5 cm x 0.5 cm x 5.0 cm producing a core sample representative of both proximal and distal airways. The strips were then dissected into small cubes of ~4 mm3. These cubes were then cultured as “explants” individually in the wells of Nunclon (Thermo Scientific, MA, USA) 12-well tissue culture plates (Nunclon Delta-treated) with shallow (~350 µl) complete stem cell culture medium (CCM) comprised of α-MEM containing 16.4% fetal bovine serum (FBS), 4 mM L-glutamine, 100 U/ml penicillin/streptomycin, and 250 ng/ml amphotericin B to facilitate attachment of the explant to the culture surface. Media changes were performed every 2–3 days. After 4–14 days of culture or when spindle-shaped cell outgrowth reached a distance of 1–2 times the diameter of the tissue explant, the explants were gently dislodged and passaged to new plates, and outgrowth cells were washed twice in PBS and passaged from the wells using TrypLE Express trypsin-free reagent (Gibco, Life Technologies, CA, USA). The outgrowth cells were expanded in CCM in Nunclon Delta-treated 15 cm cell culture dishes and cryopreserved in liquid nitrogen at passage 2 using CCM with 20% FBS and 5% DMSO as the freezing medium. Unless otherwise specified, cells were recovered in CCM overnight, diluted to an initial plating density of 200 cells/cm2, and expanded to log-phase growth for experiments.
Growth assays
To determine the colony-forming unit capacity (a basic requirement of self-renewal) of lung outgrowth cells, limiting dilutions of single-cell suspensions down to 1000 cells/ml in CCM were performed, and either 1000 cells (1 ml) or 100 cells (0.1 ml) were plated in triplicate per donor animal to 10 cm Nunclon cell culture plates in a total of 10 ml of CCM. Rhesus bone marrow-derived MSCs (BMSCs) were isolated as previously described and used in parallel as comparative controls cultured under identical conditions [22]. These plates were cultured for 2 weeks with media changes every 2–3 days. At the end of the culture period, the resulting colonies were washed twice in PBS and then fixed/stained with 3 ml per plate of 3% crystal violet in methanol for 10 minutes at room temperature. After fixation and staining, the plates were washed 3–5 times with distilled water to remove excess stain and resolve the colonies. Plates with fixed and stained colonies were air-dried at room temperature. Colonies greater than 2 mm in diameter or which contained ≥50 cells were counted. The number of colony-forming units (CFU) was determined and reported as percent CFU relative to starting cell number.
To plot the kinetics of cellular growth, lung outgrowth cells were plated at 500 cells/cm2 per well of 6-well Nunclon Delta-treated culture plates (~4800 cells per well) and cultured for 12 days in CCM. Rhesus BMSCs were used in parallel as comparative controls. Media was changed every 2–3 days. Every other day, cells in triplicate wells were trypsinized and counted. Total cell number relative to starting cell number was plotted as a function of time. Population doubling time (PDT) expressed in hours was calculated as ln(2)/growth rate; growth rate is defined as the number of doublings per unit time and was determined by exponential regression analysis.
Cell growth and migration was also analyzed in the context of a “wound healing” scratch assay. Briefly, lung explant outgrowth cells and BMSCs (both ≤P7) were cultured at ~5.0 x 105 cells per ml in the wells of Nunclon 24-well plates (2.0 cm2 surface area) for 2 hours at 37°C, 5% CO2 to allow confluent attachment of the cells to the culture surface. After washing away unattached cells with PBS, a scratch was made across the center of the growth surface using the tapered end of a 1 ml pipette tip. Images of the resulting “wound” (eight regions per sample) were taken at 0, 17, and 24 hours, and the width of the scratch was measured in five random locations per scratch using ImageJ. Results were calculated as wound width relative to the 0 hour time point.
Exponential phase L-MSC cultures were trypsinized and subjected to flow cytometric cell cycle analyses via propidium iodide stain. Using the Beckman-Coulter Epics FC500 flow cytometer running CXP software, cellular ploidy, cycle phase, G2/G1 ratio, and coefficient of variation (%CV) were determined. Estimates of cellular debris and apoptosis were also represented graphically.
Chromosome analyses
Karyotypes for lung outgrowth cells and BMSC controls were generated using the method described by Moore et al [23]. The cells were treated with 10 µl/ml of KaryoMAX Colcemid solution (Life Technologies) for 45 minutes at 37°C. Then the cells were lifted with TrypLE Express and treated with 0.075 M KCl for 10 minutes at 37°C. Next, the suspensions were fixed in Carnoy’s fixative (3:1 methanol:acetic acid) and stored at 4°C. Fixed cells were dropped onto microscope slides from a height of ~12–18 inches to burst the cells and spread the chromosomes. Air-dried slides were stained with a freshly prepared solution of Wright stain and Gurr buffer (Biomedical Specialties, CA, USA). The slides were analyzed with a light microscope at 10x and 100x magnifications.
Surface marker expression & cell cycle analyses
Lung outgrowth cells and passage-matched BMSCs and ASCs (≤P6) were expanded in culture to exponential phase growth. All cell groups were washed twice with PBS and lifted using TrypLE Express. Cell pellets were washed and resuspended in PBS to 2.0 x 106 cells/ml and filtered through 70 µm nylon mesh cell strainers. Standard human MSC staining panels and cell cycle analyses was performed by the Flow Cytometry Core Facility in the Center for Stem Cell Research and Regenerative Medicine at the Tulane University School of Medicine using the Beckman-Coulter Epics FC500 flow cytometer running CXP software. Table 1 lists the staining groups and antibodies used for the surface marker panel.
Target | Conjugate | Clone | Isotype | Manufacturer | Catalog number | Rhesus Reactivity |
---|---|---|---|---|---|---|
CD36 | FITC | FA6.152 | IgG1 | Coulter | IM0766 | + |
CD34 | PE | 581 | IgG1 | Coulter | IM1871 | + |
CD19 | ECD | J4.119 | IgG1 | Coulter | IM2708 | + |
CD11b | PeCy5 | Bear1 | IgG1 | Coulter | IM3611 | + |
CD45 | PeCy7 | J33 | IgG1 | Coulter | IM3548 | - |
PODXL | FITC | 53D11 | IgG2a | MBL | MO84-4 | ? |
CD166 | PE | 3A6 | IgG1 | Coulter | A22361 | ? |
CD90 | PeCy5 | Thy1/310 | IgG1 | Coulter | IM3703 | ? |
CD49b | FITC | Gi9 | IgG1 | Coulter | IM1425 | ? |
CD105 | PE | IG2 | IgG1 | Coulter | A07414 | ? |
CD184 | APC | 12G5 | IgG2a | BD | 555976 | ? |
CD3 | PeCy7 | UCHT1 | IgG1 | Coulter | 6607100 | - |
CD147 | FITC | HIM6 | IgG1 | BD | 555962 | ? |
CD49c | PE | C3 11.1 | IgG1 | BD | 556025 | ? |
CD29 | PeCy5 | MAR4 | IgG1 | BD | 559882 | + |
CD59 | FITC | P282E | IgG1 | Coulter | IM3456 | ? |
CD146 | PE | TEA1/34 | IgG2a | Coulter | A07483 | ? |
CD79a | PeCy5 | HM47 | IgG1 | Coulter | IM3456 | ? |
HLA-abc | FITC | G46-2.6 | IgG1 | BD | 555552 | + |
CD271 | PE | C40-1457 | IgG1 | BD | 557196 | ? |
CD49f | PeCy5 | GoH3 | IgG1 | BD | 551129 | ? |
CD117 | PeCy7 | 104D2D1 | IgG1 | Coulter | IM3698 | ? |
HLA-II | FITC | TU39 | IgG2a | BD | 555558 | + |
CD73a | PE | AD2 | IgG1 | BD | 550257 | ? |
CD106 | PeCy5 | 51-10C9 | IgG1 | BD | 551148 | ? |
HGF(cmet) | FITC | eBioclone97 | IgG1 | EBioscience | 11-8858-71 | ? |
CD49d | PE | 9F10 | IgG1 | BD | 555503 | + |
CD14 | ECD | RM052 | IgG2a | Coulter | IM2707 | + |
CD44 | APC | G44-26 | IgG2a | BD | 559942 | + |
Isotype | FITC | IgG1 | Coulter | A17599 | - | |
PE | IgG2a | Coulter | A17599 | - |
Differentiation assays
Lung outgrowth cells (less than symbol P6) were plated in triplicate to the wells of uncoated 6-well culture dishes at an initial density of 50,000 cells per plate and cultured in CCM for 1–2 days. Rhesus BMSCs of the same passage were used in parallel as comparative controls. When the cellular confluence reached 60–70%, the media were changed to either control medium (CCM), bone differentiation medium (CCM + 10 nM dexamethasone, 20 mM β-glycerolphosphate, and 50 µM L-ascorbic acid), or fat differentiation medium (CCM + 0.5 µM dexamethasone, 0.5 µM isobutylmethylxanthine, and 50 µM indomethacin). Culture media were changed every 2–3 days for 21 days. At the end of the differentiation period, the cells were analyzed for bone and fat differentiation using Alizarin Red and Oil Red-O stains, respectively. Briefly, cells in all groups were washed twice with PBS (no Ca2+/Mg2+) and fixed with 10% neutral-buffered formalin (NBF) for 30 minutes at room temperature. After fixation, bone and fat differentiation samples were washed three times with deionized water (dH2O) and PBS, respectively followed by staining with 40 mM Alizarin Red and 0.5% Oil Red-O, respectively, for 1 hour at room temperature. Bone minerals and fat droplets were resolved by washing the stained cells 3–5 times with dH2O or PBS, respectively, and viewed using standard light microscopy.
To induce chondrogenic differentiation, exponentially growing lung outgrowth cells were harvested and suspended to 1.6 x 107 cells/ml. Five microliter droplets of this cell suspension (8.0 x 104 cells) were plated in the centers of the wells of 12-well culture plates. The cells in the droplets were allowed to adhere to the plates in a humidified cell culture incubator for 2 hours after which the media was replaced with chondrogenesis medium consisting of StemPro Osteocyte/Chondrocyte Differentiation Basal Medium (Invitrogen, MA, USA) containing 1X StemPro Chondrogenesis Supplement and 2.5 ng/ml gentamicin. Fresh chondrogenesis media was replaced every 3 days for 14 days, as suggested by the manufacturer. At the end of the culture period, the cells were washed twice with PBS (no Ca2+/Mg2+) and fixed with NBF for 30 minutes at room temperature. The fixed cells were stained with 1% Alcian Blue in 0.1 N HCl for 30 minutes at room temperature followed by washing three times with 0.1 N HCl and dilution with dH2O to neutralize acidity. Cultures were analyzed by light microscopy for Alcian Blue (American Mastertech, CA, USA) staining of chondrocyte-produced glycosaminoglycans.
To better identify processes leading to adipogenic differentiation of lung outgrowth cells, total RNA isolated from control, fat, and bone differentiated samples were subjected to reverse transcription PCR (RT-PCR) targeting the glyceraldehyde 3-phosphate dehydrogenase (GAPDH) internal control gene and the adipogenesis-associated genes glucose transporter 4 (GLUT4), leptin (LEP(OB)), and peroxisome proliferator-activated receptor gamma (PPARγ). The amplified products were analyzed by electrophoresis through a 0.8% agarose gel, and the resulting band intensities were semi-quantitatively analyzed by densitometry using ImageJ.
Tube formation assay
Lung explant outgrowth cells or rhesus BMSCs were cultured at 1.25 x 105 cells/ml in 24-well plates containing ~400 μl of Matrigel (~9 mg/ml; BD, CA, USA). The cultures were held statically at 37°C, 5% CO2 to allow adhesion of the cells to the substrate followed by observation via standard light microscopy at 2, 4, and 21 hours to detect assembly and growth into tubules. For analyses of endothelial-like differentiation, L-MSCs were cultured on Matrigel at 104 cells/ml in 24-well plates containing ~400 μl of Matrigel overnight at 37°C, 5% CO2 to allow formation of tubule clusters. The cells were then incubated with 1X FITC-conjugated acetylated LDL (Ac-LDL) (Life Technologies) in CCM for 4 hours, washed three times with PBS without divalent cations, and then imaged with a fluorescent microscope to visualize uptake of Ac-LDL in the cell clusters. L-MSCs grown on tissue culture plastic were used as negative controls.
L-MSC-seeded lung matrix slice cultures
Rhesus macaque lungs were decellularized with detergents, salts, and enzymes as previously described [20]. The resulting acellular matrix was sterilized with antibiotics/antimycotics and stored at 4°C until use. Single-cell suspensions of lung outgrowth cells at 3.0 x 106 cells/ml in CCM were mixed with equal volumes of liquefied 2% SeaPrep low melting-point agarose (Lonza) at 37 °C for a final cell density of 1.5 x 106 cells/ml and a final agarose concentration of 1% in CCM. The cell-agarose suspensions were instilled into the bronchioles of intact decellularized lung lobes. After instillation, the bronchiole was clamped shut with a hemostat, and the filled lobe was submerged in ice-cold PBS for 15–20 minutes at 4°C to allow the agarose to solidify. Then, the cell-seeded tissue was cut into slices ~1–2 mm thick using a sterile scalpel. The slices were then cultured in either CCM or Small Airways Growth Medium (SAGM, Lonza Bioscience, MD, USA) in individual wells of uncoated 6-well culture plates. The slices were flipped and media were changed every other day. Samples were taken at 24 hours, 7 days, and 10 days after cell seeding and initiation of culture. Tissues were fixed in 2% paraformaldehyde and stored at 4°C. Fixed samples were embedded in paraffin, sectioned at 5 µm, stained with hematoxylin and eosin (H&E), and analyzed with an Apereo ScanScope slide-scanning light microscope. Terminal deoxynucleotidyl transferase dUTP nick end labeling (TUNEL) assays were performed on 5 µm sections of these slices using the In Situ Cell Death Detection Kit—Fluorscein (Roche Pharmaceuticals, Basel, Switzerland) according to manufacturer instructions; Proteinase K (Sigma-Aldrich) was used for fixation unmasking, and DNase I from bovine pancreas (Sigma-Aldrich) was used for the positive control.
Results
Rhesus macaque lung explants had robust spindle-shaped cell outgrowth in culture with stem cell CCM after ~4–14 days (Figure 1A–B). When the outgrowth cells produced a halo of approximately half the diameter of the explant, the explants were removed, and the adherent cells were passaged to fresh culture plastic at a density of ~500 cells/cm2; the passaged cells formed colonies of adherent cells that maintained the spindle shape of the parent population (Figure 1B). The colony-forming ability of the lung explant outgrowth cells (L-MSCs) was quantified by low-density culture for 14 days followed by crystal violet staining and colony enumeration. Compared to cultures of rhesus BMSCs of similar passages, L-MSCs demonstrated a 9.10-fold higher colony-forming capacity with 36.3 ± 13.2% CFU relative to 4.0 ± 5.6% CFU for BMSCs (Figure 2A–B). The growth kinetics of L-MSCs were characterized as having a traditional sigmoid growth curve showing lag, logarithmic, and plateau phases representing exponential growth with eventual contact inhibition (Figure 2C); however, the L-MSCs displayed rapid exponential phase growth relative to BMSC (Figure 2C open circles and inset) while the lag- and plateau phases were of similar magnitude and duration. Two-way ANOVA with replication analysis demonstrated that the growth curves of L-MSCs and BMSCs were significantly different, reaching relative cell numbers (compared to cell number at time 0) of 484.4 ± 251.0 and 77.3 ± 28.0, respectively, by 288 hours (12 days) in culture (p < 0.0001).
Cell doubling time was assessed from the growth curves of L-MSCs and BMSCs as ln(2) divided by the growth rate, where the growth rate is the number of doublings per unit time. While the growth curves displayed significantly different profiles, the doubling times based on the data in the kinetics curves were 79.9 ± 9.9 hours for L-MSCs and 66.4 ± 11.0 hours for BMSCs, and these values were not significantly different (n = 5; p = 0.074; Table 2).
Doubling Time | ||
---|---|---|
Experiment (n) | BMSCs | L-MSCs |
1 | 65.2 | 72.5 |
2 | 48.6 | 85.99 |
3 | 71.21 | 77.67 |
4 | 77.85 | 93.64 |
5 | 69.09 | 69.74 |
Mean | 66.39 | 79.908 |
Deviation | 10.95 | 9.86 |
T-test | p = 0.074 |
A wound-healing assay was performed to assess the migratory ability of the L-MSCs in response to injury mimicked by scratching the surface of a confluent culture with a 1 ml pipette tip. The mean width of the wound was measured at 0, 17, and 24 hours. In L-MSC cultures the mean relative width of the scratches was 40.0 ± 13.1% and 7.8 ± 12.5% at 17 and 24 hours, respectively, relative to the initial mean scratch width while BMSC cultures subjected to the same injury mimic had mean relative wound widths of 51.1 ± 39.9% and 38.7 ± 39.4% at 17 and 14 hours, respectively, relative to 0 hours (Figure 2D). The rate of wound closure was significantly different between the two cell groups (p = 0.001); i.e., L-MSCs were able to seal the wound more efficiently than their BMSC counterparts during the 24-hour test period.
Cell cycle analyses of exponentially growing L-MSC were performed by propidium iodide staining and flow cytometric analysis (Figure 3A). All cells analyzed were normal diploid (100.0 ± 0.0%) with 45.5 ± 11.4% of cells in G1, 8.2 ± 4.9% in G2, and 46.2 ± 6.6% in S-phase (n = 5). The G2/G1 ratio was precisely 2.0 ± 0.0, and the coefficient of variation (%CV) was 7.3 ± 0.7%. Chromosomal analyses confirmed a normal complement of 42 chromosomes for rhesus macaque cells; i.e., three female 42XX and one male 42XY for L-MSCs compared to a normal male BMSC donor with 42XY. The karyotypes of both L-MSCs and BMSCs revealed no gross chromosomal aberrations (Figure 3B).
Cell surface marker expression was analyzed by subjecting rhesus macaque L-MSCs to flow cytometric analysis using a panel of antibodies routinely used for human MSC characterization. Table 1 lists the antibodies used, manufacturer, catalog number, and clone number as well as the known cross-reactivity with rhesus spp. Note that the antibodies against CD3 and CD45 are known to be non-reactive with rhesus spp. proteins. Entries labeled with a question mark denote antibodies for which the cross-reactivity with rhesus spp. is unknown, while those labeled with plus signs are known to be reactive. Results of the analyses showed that rhesus L-MSC were positive for the cell surface markers associated with previously characterized MSCs, namely CD90, CD105, CD20, CD73, and HLA-I (Figure 4A). Conversely, the rhesus L-MSC were negative for the hematopoietic stem cell marker CD34, the granulocyte marker CD11b, and HLA-II. Note that while the results showed the cells to be negative for CD45 and CD3, it is known that these antibodies do not cross-react with rhesus cells. Quantification of the flow data showed that rhesus L-MSC expression of MSC-associated cell surface markers was robust with the exception of CD90 which was relatively low in comparison to the other MSC markers (Figure 4B). Overall, the profile of surface marker expression is consistent with that of other previously characterized MSC lines from human, macaque, and rat (Table 3) [7,8,22,24,25].
Target | Expected for human MSC (24) | Human ASC (7) | Human BMSC (7) | Mouse LMSC (8) | Rhesus BMSC (22) | Rhesus LMSC |
---|---|---|---|---|---|---|
CD36 | Negative | Positive | Negative | -- | -- | Negative |
CD34 | Negative | Negative | Negative | Negative | -- | Negative |
CD19 | Negative | Negative | Negative | -- | -- | Negative |
CD11b | Negative | Negative | Negative | Negative | Negative | Negative |
CD45 | Negative | Negative | Negative | Negative | Negative | Negative |
PODXL | -- | Negative | Positive | -- | -- | Negative |
CD166 | Positive | Positive | Positive | Negative | -- | Positive |
CD90 | Positive | Positive | Positive | Weak Positive | Positive | Weak Positive |
CD49b | Positive | -- | -- | -- | -- | Positive |
CD105 | Positive | Positive | Positive | Positive | Positive | Positive |
CD184 | Positive | Negative | Negative | -- | -- | Negative |
CD3 | -- | Negative | Negative | -- | Negative | Negative |
CD147 | Positive | Positive | Positive | -- | -- | Negative |
CD49c | Positive | Positive | Positive | -- | -- | Positive |
CD29 | Positive | Positive | Positive | -- | -- | Positive |
CD59 | Positive | Positive | Positive | -- | Positive | Positive |
CD146 | -- | Weak Positive | Positive | Positive | Positive | Positive |
CD79a | Negative | Negative | Negative | -- | -- | Negative |
HLA-abc | Positive | Positive | Positive | -- | Positive | Positive |
CD271 | Negative | Negative | Negative | -- | -- | Negative |
CD49f | Positive | Negative | Positive | -- | -- | Weak positive |
CD117 | Negative | Negative | Negative | -- | -- | Negative |
HLA-II | Negative | Negative | Negative | -- | -- | Negative |
CD73a | Positive | Positive | Positive | Positive | -- | Positive |
CD106 | Positive | Negative | Weak Positive | Positive | Positive | Positive |
HGF(cmet) | -- | Negative | Negative | -- | -- | Negative |
CD49d | -- | Positive | Weak Positive | -- | -- | Negative |
CD14 | Negative | Negative | Negative | Negative | -- | Negative |
CD44 | Positive | Positive | Positive | Positive | -- | Positive |
Culturing L-MSCs in osteogenic, adipogenic, and chondrogenic media resulted in varying degrees of cellular differentiation into bone, fat, and cartilage lineages, respectively. Both L-MSCs and their BMSC counterparts displayed robust differentiation into bone as determined by Alizarin Red staining of mineral deposits in the cells cultured with osteogenic differentiation medium (Figure 5A). After 21 days of culture in adipogenic differentiation medium, the BMSCs were noted to have copious fat droplets detected by Oil Red-O staining; however, the L-MSC showed only small lipid vesicles observable at high power magnification (200–400X). The chondrogenic cultures of L-MSCs and BMSCs both showed presence of sulfated glycans detected by Alcian blue staining, but the morphology of the resulting L-MSC cell aggregates was more compact that their BMSC counterparts and stained more darkly than the latter.
When cultured on Matrigel (~10 mg/ml) in stem cell CCM, rhesus L-MSC and BMSC assembled into tube-like networks during a 21-hour culture period (Figure 6). Moreover, L-MSC cultured overnight on Matrigel showed marginal ability to uptake Ac-LDL relative to L-MSCs cultured on standard tissue culture plastic (Supplementary Figure 1).
To further investigate the differentiation of L-MSC into adipocytes, 21-day cultures of the cells in control, adipogenic, and osteogenic medium were analyzed for mRNA expression of the adipogenic-associated genes GLUT4, OB, and PPARγ by reverse transcription PCR (Figure 5B–C). Gel electrophoresis of the amplified products with semi-quantitative densitometric analyses revealed that the cells cultured in adipogenic medium had 1.4 ± 0.3-fold, 1.3 ± 1.1-fold, and 5.0 ± 2.1-fold difference in GLUT4, OB, and PPARγ expression, respectively, relative to control media and 2.3 ± 0.5-fold, 0.4 ± 0.4-fold, and 3.2 ± 0.9-fold difference in expression of the aforementioned genes, respectively, relative to osteogenic medium. All values were normalized to the expression of GAPDH in each sample. These results indicated that gene expression pathways associated with adipogenic differentiation were activated in L-MSC cultures in adipogenic medium though terminal differentiation may not have occurred efficiently in the 21-day culture period under these conditions.
Rhesus L-MSC attached to decellularized macaque lung matrices after co-inoculation with agarose into the airway, tissue slicing, and culture in stem cell CCM and SAGM. The cells were maintained within this bioactive scaffold for up to 14 days (Figure 7). The cell-inoculated slice cultures displayed robust recellularization of alveolar parenchyma as early as 24 hours during the culture period without obliteration of luminal spaces (i.e., attachment of cells to the scaffolds appeared to be essential for survival) (Figure 7A). Cellular morphology was similar between CCM and SAGM cultures; however, at later time points (notably at 14 days), there was evidence of nuclear blebbing and fragmentation consistent with apoptosis in the SAGM group (Figure 7B, arrows). These changes were not noted in the CCM cultured samples. Increased apoptosis in SAGM cultured slices was confirmed by TUNEL staining and represented as percent TUNEL-positive cells per 100X field (Figure 8). Apoptosis in the 14-day SAGM slice cultures was 5.0 ± 4.4-fold, 6.4 ± 5.5-fold, and 5.0 ± 3.0-fold greater than the CCM 24 hour, CCM 14 day, and SAGM 24 hour groups, respectively. Tukey-Kramer analysis showed that this increased incidence of apoptosis in SAGM cultures at 14 days was significantly greater than all other culture groups at all time points.
Conclusions
We conclude that plastic-adherent, spindle-shaped outgrowth cells obtained from rhesus macaque lung explants are likely a population of L-MSCs. These cells have the potential to aide in the recellularization of decellularized lung matrices for the purpose of engineering bioartificial pulmonary tissues.
Discussion
This report details the isolation and initial characterization of tissue-resident multipotent mesenchymal stromal cells in the lungs of rhesus macaques. Tissue-resident mesenchymal stem cells have been isolated from a variety of species by different means including explant culture [2,8,26], plating of adherent cells from bronchioalveolar lavage or digested tissue [4,9,12], and flow cytometry of single-cell suspensions with FACS [10]. Identification of sub-populations of candidate cells in lung extracts by Hoechst vital dye efflux with negative expression of hematopoietic and endothelial markers has produced lines of multipotent mesenchymal-like cells which behave like other MSCs; the same is true of side-population cells isolated in a similar manner using Hoechst in lung [11] and bone marrow, the latter of which has been shown to contribute to lung and liver cellular constituents [27]. The explant culture method used in this report results in the isolation of a heterogeneous population of fibroblast-like cells that display compelling characteristics similar to MSCs isolated from bone marrow and adipose. This method provides a high-throughput avenue through which large numbers of cells can be isolated easily with little expense and time commitment. The various constituents of this lung-resident MSC population are currently unknown, and further experiments are required to characterize this heterogeneity. Also unknown is the in situ reservoir for this cell population. In murine lungs, the Sca-1+ cell fraction has been shown to be enriched with fibroblastic progenitor cells, suggesting that these progenitors harbor some stem-like capacity [28]. Full characterization of these cells and their subpopulations will allow a better understanding of their physiological function and may lead to clues as to how these cells can be manipulated for therapeutic benefit.
Rhesus macaque L-MSCs had ~9-fold higher CFU efficiency than the bone marrow-derived MSCs; moreover, their exponential phase growth and wound closure (migratory) ability were significantly elevated. Hoffman et al noted increased CFU efficiency of murine L-MSC isolated by a similar explant culture method [8]. While moderately variable, rhesus L-MSC cultures displayed some degree of kinetic plateau consistent with contact inhibition or other growth arrest around 240 hours in culture; this finding was consistent with the timing of growth plateau in rhesus BMSCs. Even with the increased rate of growth in exponential phase, the overall doubling times for L-MSC and BMSCs were not significantly different. Elevated growth rates of cultured cells can be indicative of neoplastic transformation; however, we noted that all cells analyzed were diploid with normal progression through the cell cycle. In addition, our chromosomal analyses showed no aneuploidy or other changes consistent with transformation.
There was some limitation to our flow cytometric characterization of rhesus L-MSCs in that we used a panel of antibodies routinely employed for human MSC characterization. Given the genetic similarity between humans and NHPs, a broad spectrum of cross-reactivity between human-specific antibodies and NHP antigens likely exists. Notably, it is known that the antibodies we used against CD45 and CD3 have no cross-reactivity with rhesus species (Beckman-Coulter, CA, USA). As such, expression data for these antigens was negative by our tests; however, we were able to conclude that expression of other hematopoietic markers for which our antibodies were known to cross-react (i.e., CD34, CD11b, CD19), was negative in this rhesus L-MSC population. While the L-MSCs were also negative for other hematopoietic markers including CD184, CD79a, and CD117, it is unknown whether the antibodies used have reactivity in rhesus. For positive reactions with antibodies of unknown rhesus reactivity, we conclude that the cells do, in fact, express those particular cell surface markers. CD90 expression in rhesus L-MSCs was relatively low compared to previously characterized rhesus bone marrow- and adipose-derived MSCs as well as human MSCs [29]. While the reactivity of the CD90 antibody with rhesus cells is unknown, we note that a similarly low-level expression of this protein was observed in the murine L-MSC isolated by Hoffman et al [8]. Additionally, it is known that CD90 (Thy-1) in lung epithelial cells is involved in alveolar development [30]; however, it is not clear as to whether expression of this protein in the supporting pulmonary stroma is required for such processes.
L-MSCs and BMSCs from rhesus macaques have different capabilities of osteogenic, adipogenic, and chondrogenic differentiation. We showed that differentiation of rhesus L-MSCs in vitro demonstrated robust propensity of the cells to differentiate into bone when cultured under the previously described conditions for their BMSC counterparts. However, while the rhesus BMSCs produced copious Oil Red-O-positive lipid droplets in culture with fat differentiation medium, the L-MSC showed only small, vesicular Oil Red-O-positive structures. Although we showed that adipogenic genes are upregulated in the L-MSC adipogenic differentiation cultures, further optimization of the necessary culture conditions must be done in order to examine the full potential of these cells to differentiate into mature adipocytes. Similarly, the optimal chondrogenic culture condition for L-MSCs must be determined empirically; these experiments are the subject of ongoing investigation in our laboratory. Other than the traditional tri-lineage differentiation usually analyzed in MSC populations, the potential of tissue-resident cells to contribute to the functional cellularity of the home tissue should be investigated. Engraftment of distant-site MSC (bone marrow) in the lung and subsequent differentiation into airway epithelia has been documented [31]; however, the efficiency of engraftment is extremely low [32,33]. Nevertheless, there is still a possibility that locally residing stromal cell populations contribute to the epithelial or endothelial cell constituents of lungs or at least aid in natural epithelial turnover. Demonstration of this phenomenon would have tremendous implications for therapeutic interventions for pulmonary diseases. In this initial study, the reported isolated L-MSC population showed a distinct tube-like network formation, and exhibited the ability to uptake Ac-LDL when cultured on Matrigel substrate. Similar findings have been reported previously with MSCs self-assembling into tube-like networks on Matrigel substrate [34–36]. A follow-up study characterizing the formation of true tubules with hollow lumen, tube lengths and tube count should be performed to further elucidate the L-MSC cell population ability to form such capillary-like structure as compared to MSCs. While future work would have to be done to demonstrate true endothelial differentiation of these cells, the results we obtained in this study provide encouraging evidence that L-MSCs may have a wide spectrum of novel use in downstream applications. Previous reports have shown that MSCs enhance the tube-forming ability of endothelial cells, and this may be a key use of these lung-derived stem-like cells in tissue engineering applications where blood vessels must be regenerated (vasculogenesis) or re-populated (vascular scaffold seeding) [37,38].
Given the supportive role of the mesenchyme in organogenesis, it is reasonable to propose that mesenchymal cells will be important for engineering pulmonary tissue that is as close to the native condition as possible. In our recent paper, the initial interaction of rhesus bone marrow- and adipose-derived mesenchymal stem cells with decellularized rhesus lung matrices was described [20]. Here, it was determined that lung-derived MSCs from rhesus macaques can be cultured in the environment of decellularized lung matrices without overgrowth or occlusion of airway structures, and they can be maintained in simple culture media until subsequent seeding of endothelial or epithelial cells or until differentiation of the MSCs occurs; the latter being an unlikely scenario as this phenomenon has yet to be adequately described. Culture in SAGM appeared to be hazardous to the L-MSC population in the context of the lung matrix; this finding reinforces the question of whether they will be able to enhance the engraftment and differentiation of mature pulmonary epithelial cells which will likely require refined airway-specific culture media. Further studies should investigate the effect of different media formulations on the L-MSC population and whether these L-MSCs have an effect on candidate pulmonary cell populations mediated by soluble factors or direct contact.
Translational Insight
There are an estimated 1,500 individuals waiting for a lung transplant in the USA at any given time. Identifying alternatives to traditional organ transplantation represents a significant unmet clinical need. In tissue engineering and regenerative medicine there is on going research focused on the identification of the most effective cell type(s) to cellularize tissue scaffolds, such as decellularized whole-lungs.
For pulmonary tissue engineering, recellularization of decellularized lung scaffolds with pulmonary epithelial and endothelial cells may be enhanced by co-seeding lung-derived multipotent stromal cells; future studies will focus on the potentiation of the pulmonary phenotype in epithelial cells co-cultured with L-MSCs. Concomitantly, we will assess the effect of L-MSCs on endothelial cell phenotype and function.
Figures
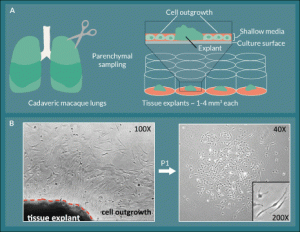
Figure 1. L-MSC are isolated by collecting lung explant outgrowth cells.
A) Small explants were excised from cadaveric macaque lung tissue and plated in the centers of the wells of 12-well tissue culture plates
in shallow medium. B) Between 4–14 days, spindle-shaped outgrowth cells were observed in a halo-like fashion attached to the plate
around the explant. The outgrowth cells were collected using trypsin-free reagents and passaged onto fresh culture plates where they
retained their spindle-shaped morphology and formed colonies
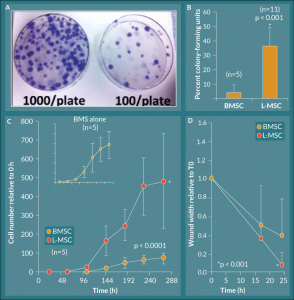
Figure 2. L-MSC exhibit rapid exponential growth and increased migratory wound closure ability. A) Lung explant outgrowth cells (L-MSC) efficiently formed colonies on 10 cm tissue culture plates over a 14 day period, and this effect was titrated by seeding fewer cells per plate at the initial culture. B) The culture forming ability of lung explant outgrowth cells was quantified as percent CFU; L-MSC formed colonies significantly more efficiently relative to their BMSC counterparts (p < 0.001, n = 5 BMSC, n = 11 L-MSC). C) Growth kinetics were plotted as cell number relative to initial seeding number (0 hours). L-MSC displayed a robust exponential phase but maintained a standard growth curve with lag, log, and plateau phases. BMSC controls displayed the same profile albeit with significantly less cell numbers achieved overall (p < 0.0001, n = 5). D) L-MSC showed significantly increased ability to migrate and close artificial wounds generated in tissue culture as compared to their BMSC counterparts (p = 0.001).
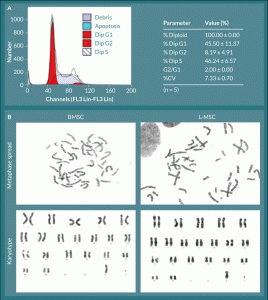
Figure 3. L-MSC have normal cell cycle progression and no visible chromosomal aberrations.
A) All L-MSC were found to be normal diploid cells with normal progression through the cell cycle. B) L-MSC and BMSC were analyzed
for chromosome number and arrangement; all cells, regardless of origin, displayed normal rhesus macaque complements of 42
chromosomes (20 pairs of autosomes and 1 pair of allosomes—XX or XY). Representative male karyotypes are shown for each cell type.
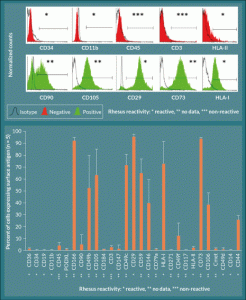
Figure 4. Surface marker expression in L-MSC is consistent with previously characterized MSC types. A) L-MSC are negative for hematopoietic markers and HLA class II (note that there is no reactivity of the CD45 and CD3 antibodies used with rhesus macaque), but the cells are positive for markers associated with mesenchymal stem cells (CD90, CD105, CD29, CD73, HLA-I). B) Expression levels of each marker were quantified using the forward and side scatter profiles and are expressed as percent of cells (events) expressing the given antigen (n = 5).
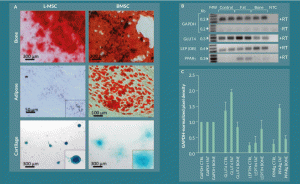
Figure 5. L-MSC can be differentiated into other mesenchymal lineages but with less efficiency than BMSC under standard conditions. A) L-MSCs and BMSCs were cultured in control, osteogenic, adipogenic, and chondrogenic differentiation media for 21 days followed by fixation and staining with Alizarin Red, Oil Red-O, and Alcian Blue for bone, fat, and cartilage, respectively. Bone differentiation was copious for both L-MSCs and BMSCs. BMSCs produced large fat droplets while L-MSCs only showed very small Oil Red-O positivity in indistinct granules. Both cell types stained positive for Alcian Blue in chondrogenic cultures; however, the L-MSC organoids had a more compact, densely stained morphology than their BMSC counterparts. B) Adipogenic differentiation potential of the L-MSC fat-differentiated cultures was investigated by reverse transcription PCR. GLUT4 and PPAR γ showed increased expression when the amplified products were subjected to gel electrophoresis, and C) this observation was quantified by densitometry.
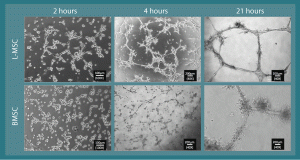
Figure 6. L-MSC have tube-forming ability. When cultured on Matrigel (~10 mg/ml), L-MSC assemble into alveolarized tubules within a 24 hour period. A similar phenomenon was observed in rhesus BMSC. The intermediate observations at 4 hours show the intercellular connections commencing.
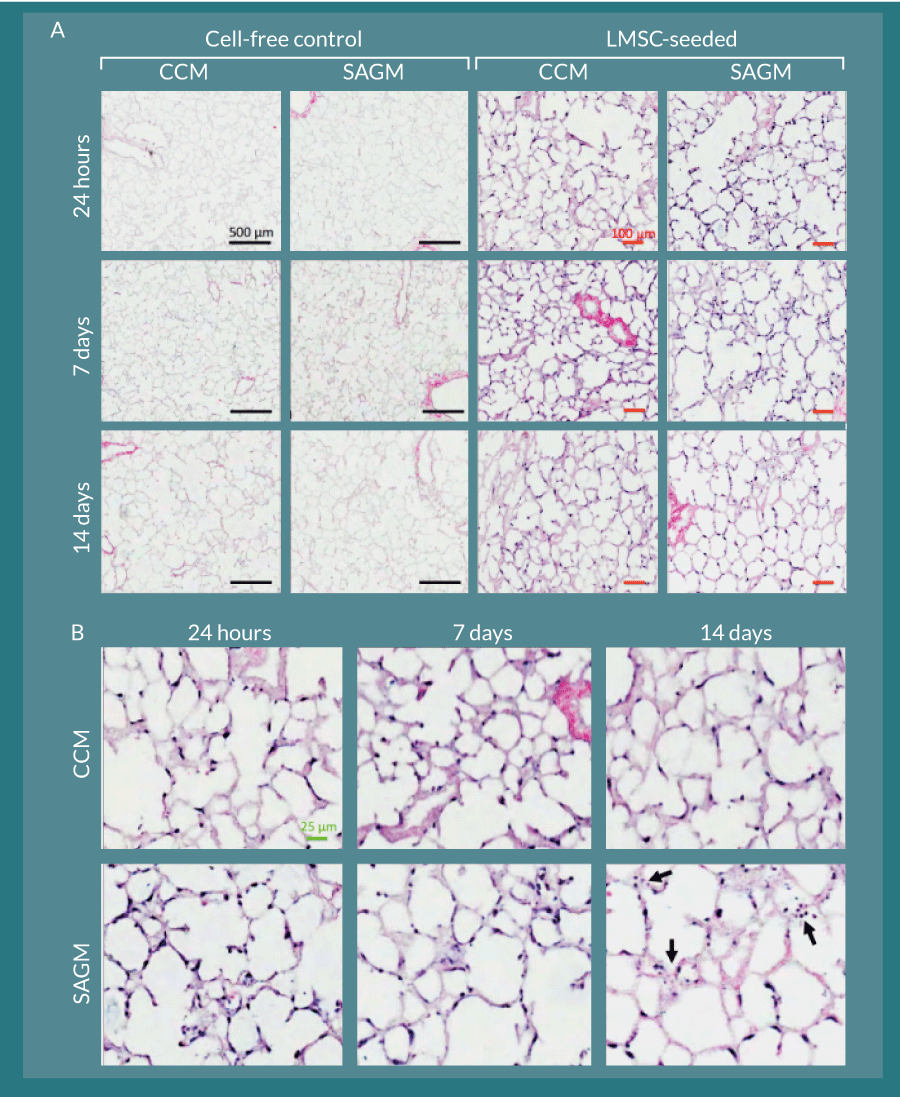
Figure 7. L-MSC can attach to and persist within decellularized rhesus lung scaffolds. A) Cell-free control slice cultures displayed no cellular growth during the two-week culture period. L-MSC-seeded scaffolds displayed robust and even cellular coverage throughout the culture period in both CCM- and SAGM-cultured slices. Black bars represent 500 µm; red bars represent 100 µm. B) Magnified images of the L-MSC seeded scaffold slices show various cellular orientations and morphologies along the decellularized alveolar septae. By 14 days, there was some evidence of nuclear fragmentation consistent with apoptosis in the SAGM cultured slices (arrows). The green bar represents 25 µm.
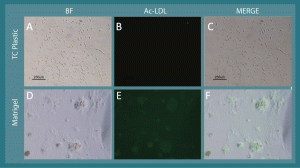
Supplementary Figure 1. L-MSC take up acetylated LDL after tube-like network formation via culture on Matrigel. A) When cultured on virgin tissue culture plastic, L-MSC have a fibroblastoid morphology, and B) they fail to uptake FITC-conjugated acetylated LDL. Conversely, when cultured on Matrigel, the cells form tube-like networks (D) , and FITC-acetylated LDL is taken up by the network clusters (E). C and F represent merged images from brightfield and FITC fluorescence micrographs; the localization of FITC-acetylated LDL is consistent with the cellular networks.
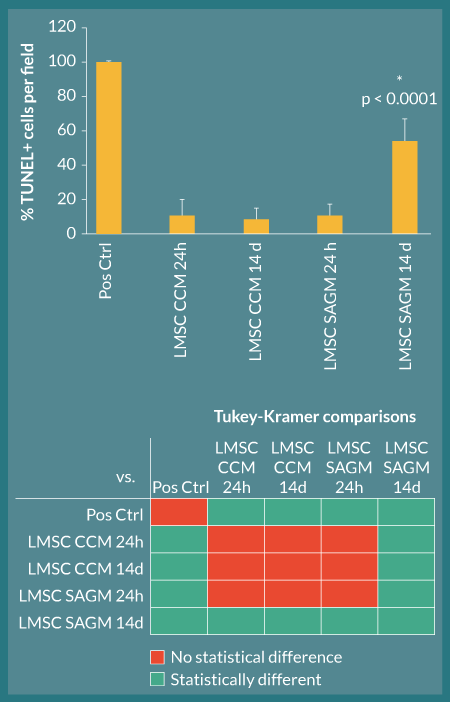
Figure 8. L-MSC apoptosis is increased when cell-seeded lung scaffolds are cultured in SAGM. TUNEL analysis was used to quantify apoptosis occurring in L-MSC-seeded decellularized rhesus lung scaffolds. All culture groups showed low apoptosis (baseline) at 24 hours in culture; however, by 14 days, the SAGM-cultured slices had significantly more apoptotic cells per field than their CCM-cultured counterparts (asterisk; ANOVA p < 0.0001 with Tukey-Kramer post-hoc comparisons); these quantifications are consistent with qualitative histological observations. Below the graph, colored squares in each row denote the groups being compared by Tukey-Kramer analyses; red squares represent no statistical significance and green squares represent statistical significance at α=0.05 (e.g., in comparison 1, Pos Ctrl and LMSC CCM 24H are being compared and are significantly different)
financial & competing interests disclosure
The first and corresponding authors do not have any disclosures. They do not have any relevant financial involvement with an organisation or entity with a financial interest in or financial conflict with the subject matter or materials discussed in the manuscript. This includes employment, consultancies, honoraria, stock options or ownership, expert testimony, grants or patents received or pending, or royalties.
No writing assistance was utilised in the production of this manuscript.
A Betancourt is the co-founder, co-owner and Chief Scientific Officer of Wibi+Works Therapeutics.
ETHICAL CONDUCT OF RESEARCH
The authors state that they have obtained appropriate institutional review board approval or have followed the principles outlined in the Declaration of Helsinki for all human or animal experimental investigations. In addition, for investigations involving human subjects, informed consent has been obtained from the participants involved.
ACKNOWLEDGEMENTS
The authors would like to thank H Alan Tucker, Dina Gaupp, and Claire Llamas of the flow cytometry and histology core laboratories at the Center for Stem Cell Research and Regenerative Medicine at the Tulane University School of Medicine. Much appreciated are the efforts of Cynthia Trygg in the Division of Regenerative Medicine and Maurice Duplantis in the Department of Pathology at the Tulane National Primate Research Center in procuring rhesus macaque tissues. Nicholas C. Pashos is supported by an NSF IGERT training grant in Bioinnovation, DGE-1144646.
REFERENCES
1. da Silva Meirelles L, Chagastelles P, Nardi N, Mesenchymal stem cells reside in virtually all post-natal organs and tissues. J. Cell Sci. 2006; 119(11), 2204–13. CrossRef
2. You S, Kublin C, Avidan O, Miyasaki D, Zoukhri D. Isolation and propagation of mesenchymal stem cells from the lacrimal gland. Invest. Ophthalmol. Vis. Sci. 2011; 52(5), 2087–94. CrossRef
3. Kotton DN, Fine A. Lung stem cells. Cell Tissue Res. 2008; 331(1),145–56. CrossRef
4. Brandau, S. Jakob M, Hemeda H, Bruderek K, Janeschik S, Bootz F, Lang S. Tissue-resident mesenchymal stem cells attract peripheral blood neutrophils and enhance their inflammatory activity in response to microbial challenge. J. Leukoc. Biol. 2010; 88(5), 1005–15. CrossRef
5. Horwitz EM, Le Blanc K, Dominici M et al. Clarification of the nomenclature for MSC: The International Society for Cellular Therapy position statement. Cytotherapy 2005; 7(5), 393–5. CrossRef
6. Kolf CM, Cho E, Tuan RS. Mesenchymal stromal cells. Biology of adult mesenchymal stem cells: regulation of niche, self-renewal and differentiation. Arthritis Res. Ther. 2007; 9(1), 204. CrossRef
7. Pachón-Pe-a G, Yu G, Tucker A, et al. Stromal stem cells from adipose tissue and bone marrow of age-matched female donors display distinct immunophenotypic profiles. J. Cell Physiol. 2011; 226(3), 843–51. CrossRef
8. Hoffman AM, Paxson JA, Mazan MR, et al. Lung-derived mesenchymal stromal cell post-transplantation survival, persistence, paracrine expression, and repair of elastase-injured lung. Stem Cells Dev. 2011; 20(10), 1779–92. CrossRef
9. Lama VN, Smith L, Badri L et al. Evidence for tissue-resident mesenchymal stem cells in human adult lung from studies of transplanted allografts. J Clin. Invest. 2007; 117(4), 989–96. CrossRef
10. Summer R, Fitzsimmons K, Dwyer D, Murphy J, Fine A. Isolation of an adult mouse lung mesenchymal progenitor cell population. Am. J. Respir. Cell Mol. Biol. 2007; 37(2), 152–9. CrossRef
11. Martin J, Helm K, Ruegg P, Varella-Garcia M, Burnham E, Majka S. Adult lung side population cells have mesenchymal stem cell potential. Cytotherapy, 2008; 10(2), 140–51. CrossRef
12. Ricciardi M, Malpeli G, Bifari F et al. Comparison of epithelial differentiation and immune regulatory properties of mesenchymal stromal cells derived from human lung and bone marrow. PLoS One, 2012; 7(5), e35639. CrossRef
13. Jarvinen L, Badri L, Wettlaufer S et al. Lung resident mesenchymal stem cells isolated from human lung allografts inhibit T cell proliferation via a soluble mediator. J. Immunol. 2008; 181(6), 4389–96. CrossRef
14. Jun D, Garat C, West J et al. The pathology of bleomycin-induced fibrosis is associated with loss of resident lung mesenchymal stem cells that regulate effector T-cell proliferation. Stem Cells 2011; 29(4), 725–35. CrossRef
15. Hogan BL and Yingling JM. Epithelial/mesenchymal interactions and branching morphogenesis of the lung. Curr. Opin Genet. Dev. 1998; 8(4), 481–6. CrossRef
16. Spooner BS and Wessells NK. Mammalian lung development: interactions in primordium formation and bronchial morphogenesis. J. Exp. Zool. 1970; 175(4), 445–54. CrossRef
17. Wessells, NK. Mammalian lung development: interactions in formation and morphogenesis of tracheal buds. J. Exp. Zool. 1970; 175(4), 455–66. CrossRef
18. Sinclair K, Yerkovich S, Chambers DC. Mesenchymal stem cells and the lung. Respirology, 2013; 18(3), 397–411. CrossRef
19. Walker N, Badri L, Wettlaufer S et al. Resident tissue-specific mesenchymal progenitor cells contribute to fibrogenesis in human lung allografts. Am. J. Pathol, 2011; 178(6), 2461–9. CrossRef
20. Bonvillain RW, Danchuk S, Sullivan DE et al. A Nonhuman Primate Model of Lung Regeneration: Detergent-Mediated Decellularization and Initial In Vitro Recellularization with Mesenchymal Stem Cells. Tissue Eng. Part A, 2012; 18(23–24), 2437–52. CrossRef
21. Bonvillain, RW, Scarritt ME, Pashos NC et al. Nonhuman primate lung decellularization and recellularization using a specialized large-organ bioreactor. J. Vis. Exp. 2013; (82), e50825. CrossRef
22. Izadpanah R, Joswig T, Tsien F, Dufour J, Kirijan JC, Bunnell BA. Characterization of multipotent mesenchymal stem cells from the bone marrow of rhesus macaques. Stem Cells Dev. 2005; 14(4), 440–51. CrossRef
23. Moore CM, Janish C, Eddy CA, Hubbard GB, Leland MM, Rogers J. Cytogenetic and fertility studies of a rheboon, rhesus macaque (Macaca mulatta) x baboon (Papio hamadryas) cross: further support for a single karyotype nomenclature. Am. J. Phys. Anthropol. 1999; 110(2), 119–27. CrossRef
24. Izadpanah R, Trygg C, Patel B, et al. Biologic properties of mesenchymal stem cells derived from bone marrow and adipose tissue. J. Cell Biochem. 2006; 99(5), 1285–97. CrossRef
25. Reger R, Tucker AH, Wolfe MR. Differentiation and Characterization of Human MSCs. Methods Mol. Biol. 2008; 449, 93–107. CrossRef
26. Ingenito, EP, Tsai L, Murthy S, Tyagi S, Mazan M, Hoffman A. Autologous lung-derived mesenchymal stem cell transplantation in experimental emphysema. Cell Transplant, 2012; 21(1), 175–89. CrossRef
27. Abe S, Lauby G, Boyer C, Rennard SI, Sharp JG. Transplanted BM and BM side population cells contribute progeny to the lung and liver in irradiated mice. Cytotherapy, 2003; 5(6), 523–33. CrossRef
28. McQualter JL, Brouard N, Williams B et al. Endogenous fibroblastic progenitor cells in the adult mouse lung are highly enriched in the sca-1 positive cell fraction. Stem Cells, 2009; 27(3), 623–33. CrossRef
29. Dominici G, Bellavite P, di Stanislao C, Gulia P, Pitari G. Double-blind, placebo-controlled homeopathic pathogenetic trials: symptom collection and analysis. Homeopathy, 2006; 95(3), 123–30. CrossRef
30. Nicola T, Hagood JS, James ML et al. Loss of Thy-1 inhibits alveolar development in the newborn mouse lung. Am. J. Physiol. Lung Cell Mol. Physiol. 2009; 296(5), L738–50. CrossRef
31. Wang G, Bunnell BA, Painter RG et al. Adult stem cells from bone marrow stroma differentiate into airway epithelial cells: potential therapy for cystic fibrosis. Proc. Natl Acad. Sci. USA. 2005; 102(1), 186–91. CrossRef
32. Kotton DN, Fabian AJ, Mulligan RC. Failure of bone marrow to reconstitute lung epithelium. Am. J. Respir. Cell Mol. Biol. 2005; 33(4), 328–34. CrossRef
33. Weiss DJ. Stem cells, cell therapies, and bioengineering in lung biology and diseases. Comprehensive review of the recent literature 2010–2012. Ann. Am. Thorac Soc. 2013; 10(5), S45–97. CrossRef
34. Bagley RG, Weber W, Rouleau C et al. Human mesenchymal stem cells from bone marrow express tumor endothelial and stromal markers. Int. J. Oncol. 2009; 34(3), 619–27. CrossRef
35. Portalska KJ, Leferink A, Groen N et al. Endothelial differentiation of mesenchymal stromal cells. PLoS One. 2012; 7(10), e46842. CrossRef
36. Zhang T, Lee YW, Rui YF, Cheng TY, Jiang XH, Li G. Bone marrow-derived mesenchymal stem cells promote growth and angiogenesis of breast and prostate tumors. Stem Cell Res. Ther. 2013; 4(3), 70. CrossRef
37. Ott HC, Clippinger B, Conrad C et al. Regeneration and orthotopic transplantation of a bioartificial lung. Nat. Med. 2010; 16(8), 927–33. CrossRef
38. Petersen TH, Calle EA, Zhao L et al. Tissue-engineered lungs for in vivo implantation. Science 2010; 329(5991), 538–41. CrossRef
Affiliations
Ryan W Bonvillain1, Michelle E Scarritt1, Nicholas C Pashos1,2, Deborah E Sullivan1,3, Aline M Betancourt1,3, Fern Tsien4, Ayesha P Umrigar4, Andrew M Hoffman5 and Bruce A Bunnell1,6*
1Center For Stem Cell Research and Regenerative Medicine, , Tulane University School of Medicine, New Orleans, LA 70112
2Bioinnovation PhD Program, Tulane University, School of Science and Engineering, New Orleans LA 70118
3Department of Microbiology and Immunology, Tulane University School of Medicine, New Orleans, LA 70112
4Department of Genetics, Louisiana State University Health Sciences Center, New Orleans, LA 70112
5Department of Clinical Sciences, Cummings School of Veterinary Medicine, Tufts University, North Grafton, MA 01536
6Department of Pharmacology, Tulane University School of Medicine, New Orleans, LA 70112
†United Therapeutics Corporation, Research Triangle Park, NC 27709
*Corresponding Author:Bruce A Bunnell, PhD, Professor and Director, Center for Stem Cell Research and Regenerative Medicine, Department of Pharmacology, Tulane University School of Medicine, 1430 Tulane Avenue, SL-99, New Orleans, LA 70112.
Phone: +1 (504) 988–7711.
Fax: +1 (504) 988–7710.
Email: bbunnell@tulane.edu